A Brief Review of Methods and Requirements
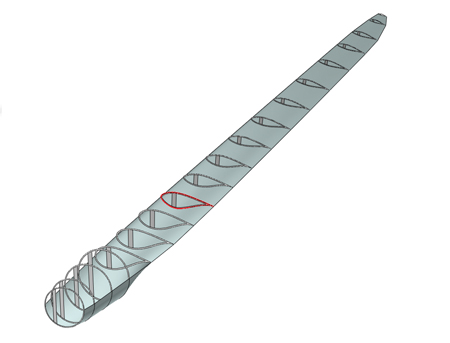
By Joseph Youssif Saab Jr and Alexandre Martuscelli Faria, Brazil
In the following paragraphs, a selection of relevant research aiming to develop horizontal-axis wind turbine (HAWT) blade airfoil geometries over the past 20 years is presented and briefly discussed, with emphasis on development methods and early design-for-noise measures, whenever adopted.
Guidati and Wagner (2000)
A three-step noise prediction method was set up by Guidati and Wagner in order to study the influence of the turbulent boundary layer structure on the blade airfoil trailing-edge (TE) noise and to investigate airfoil shape influence on noise emission. The first step of the method employed the XFOIL code to compute the flow around the airfoil. The second step was to reconstruct the TE velocity profile, and the last step was to obtain the far-field noise by employing the TNO TE noise model. One important outcome of that research was the comparison of the XFOIL model against data measured at the Laminar Wind Tunnel of the Institute of Aerodynamics and Gas Dynamics of the University of Stuttgart, in the form of drag polar curves for the different airfoil profiles tested, with favourable results. The main conclusion was that a moderate noise reduction was achievable by airfoil shape manipulation.
Fuglsang and Bak (2004)
Fuglsang and Bak employed an airfoil direct design method with an optimisation process coupled with the XFOIL code. The design variables were the control points of the curves that described the airfoil shape. The solution method was considered robust, even if computationally expensive, and that resulted in the RISØ family of airfoils. The optimisation problem did not consider minimising TE noise as part of the objective function or as a constraint. The available design data for the RISØ family of airfoils is shown in Table 1.
Fuglsang and Bak employed an airfoil direct design method with an optimisation process coupled with the XFOIL code. The design variables were the control points of the curves that described the airfoil shape. The solution method was considered robust, even if computationally expensive, and that resulted in the RISØ family of airfoils. The optimisation problem did not consider minimising TE noise as part of the objective function or as a constraint. The available design data for the RISØ family of airfoils is shown in Table 1.
Bertagnolio et al. (2009)
Despite the fact that Bertagnolio et al. concluded that the TNO model for TE noise was under-predicting the noise by up to 10dB when compared with the NACA0012 data measured by NASA and at the Aeroacoustic Wind Tunnel Braunschweig, the authors considered the model useful for relative performance comparison of airfoils. They then coupled it to an optimisation code developed at RISØ in order to reduce a cost function subjected to many constraints. Basically, the code calculated the local gradient of the cost function associated with each airfoil parameter, adopting values that would minimise the cost function. The treatment to avoid local minimum values is not described. The RISØ-B1-18 airfoil produced by the method was subjected to optimisation at a single angle of attack of 6 degrees. The authors concluded that the optimised design showed a reduction of noise in the range of 1 to 2dB compared with the baseline airfoil.
Despite the fact that Bertagnolio et al. concluded that the TNO model for TE noise was under-predicting the noise by up to 10dB when compared with the NACA0012 data measured by NASA and at the Aeroacoustic Wind Tunnel Braunschweig, the authors considered the model useful for relative performance comparison of airfoils. They then coupled it to an optimisation code developed at RISØ in order to reduce a cost function subjected to many constraints. Basically, the code calculated the local gradient of the cost function associated with each airfoil parameter, adopting values that would minimise the cost function. The treatment to avoid local minimum values is not described. The RISØ-B1-18 airfoil produced by the method was subjected to optimisation at a single angle of attack of 6 degrees. The authors concluded that the optimised design showed a reduction of noise in the range of 1 to 2dB compared with the baseline airfoil.
Cheng et al. (2016)
Cheng et al. coupled numerical optimisation to the flow solver RFOIL and also employed a genetic algorithm method in order to perform the optimisation of a wind turbine (WT) blade design by adapting and morphing a ‘shape function’. This study resulted in a family of three airfoils, which were described as the ‘WT’ series, with relative thicknesses in the range of 15 to 21%. Their study compares the newly developed WT-180 airfoil with some selected benchmarks (NREL-S810 and the NACA-63-418) in the RFOIL environment, with the WT-180 displaying higher aerodynamic efficiency at the design flow conditions. A similar RFOIL analysis was run for the WT-150 and WT-201 airfoils, but only the WT-180 was later subjected to wind tunnel testing, which basically agreed with the RFOIL analysis. No acoustic design constraints were considered; however, the authors prescribed a sharp TE in order ‘to prevent excessive noise’.
Cheng et al. coupled numerical optimisation to the flow solver RFOIL and also employed a genetic algorithm method in order to perform the optimisation of a wind turbine (WT) blade design by adapting and morphing a ‘shape function’. This study resulted in a family of three airfoils, which were described as the ‘WT’ series, with relative thicknesses in the range of 15 to 21%. Their study compares the newly developed WT-180 airfoil with some selected benchmarks (NREL-S810 and the NACA-63-418) in the RFOIL environment, with the WT-180 displaying higher aerodynamic efficiency at the design flow conditions. A similar RFOIL analysis was run for the WT-150 and WT-201 airfoils, but only the WT-180 was later subjected to wind tunnel testing, which basically agreed with the RFOIL analysis. No acoustic design constraints were considered; however, the authors prescribed a sharp TE in order ‘to prevent excessive noise’.
Grasso (2016)
Grasso reported on the development and wind tunnel testing of a family of airfoils developed for large WTs. The development was accomplished via multidisciplinary design optimisation founded on a gradient-based algorithm. The airfoil was represented by parametric curves, with 13 control points, and the aerodynamic behaviour was evaluated via RFOIL code. The objective function is a linear, weighted combination of the aerodynamic efficiency and sectional moment of inertia. A family of airfoils designated ECN-G1-XX was designed with relative thicknesses ranging from 15 to 21%. The ECN-G1-21 airfoil model was tested in a wind tunnel at very low turbulence intensity. The stall behaviour was more sudden than predicted but the pressure distributions were correctly predicted by RFOIL. In order to improve the stall characteristics, a post-stall coefficient of lift drop constraint was added, resulting in the ECN-G3-XX. It showed smoother stall characteristics with the penalty of reducing the aerodynamic efficiency.
Grasso reported on the development and wind tunnel testing of a family of airfoils developed for large WTs. The development was accomplished via multidisciplinary design optimisation founded on a gradient-based algorithm. The airfoil was represented by parametric curves, with 13 control points, and the aerodynamic behaviour was evaluated via RFOIL code. The objective function is a linear, weighted combination of the aerodynamic efficiency and sectional moment of inertia. A family of airfoils designated ECN-G1-XX was designed with relative thicknesses ranging from 15 to 21%. The ECN-G1-21 airfoil model was tested in a wind tunnel at very low turbulence intensity. The stall behaviour was more sudden than predicted but the pressure distributions were correctly predicted by RFOIL. In order to improve the stall characteristics, a post-stall coefficient of lift drop constraint was added, resulting in the ECN-G3-XX. It showed smoother stall characteristics with the penalty of reducing the aerodynamic efficiency.
Saab et al. (2018)
This development was aimed at larger size blades with rotors in the 100-metre diameter class and more realistic operating conditions. The authors employed the open source platform QBlade, which integrates the XFOIL flow solver with a three-dimensional rotor Blade Element Momentum module and the PNoise TE Noise Prediction code developed at the Polytechnic School of the University of São Paulo (Poli-USP) in order to design high performance airfoils with controlled TE noise emission. The ‘SP’ family of airfoils emerged with good theoretical aerodynamic and aeroacoustic performance increases when compared with the baseline S830 airfoil. The airfoils are listed in Table 1 and their sets of coordinates may be downloaded from popular airfoil databases on the web for research.
This development was aimed at larger size blades with rotors in the 100-metre diameter class and more realistic operating conditions. The authors employed the open source platform QBlade, which integrates the XFOIL flow solver with a three-dimensional rotor Blade Element Momentum module and the PNoise TE Noise Prediction code developed at the Polytechnic School of the University of São Paulo (Poli-USP) in order to design high performance airfoils with controlled TE noise emission. The ‘SP’ family of airfoils emerged with good theoretical aerodynamic and aeroacoustic performance increases when compared with the baseline S830 airfoil. The airfoils are listed in Table 1 and their sets of coordinates may be downloaded from popular airfoil databases on the web for research.
Miller et al. (2018)
Miller et al. presented a new family of flatback airfoils created by optimisation through a genetic algorithm in which the airfoil shapes were modified using curve parameterisation. The aerodynamic performance of the family of airfoils was evaluated with the aid of XFOIL code, and structural analysis was also considered in this case. Flatback airfoils may be found in the inner-to-mid region of the WT blades. They usually have a high resistance to flapwise bending and may attain high maximum lift coefficients. Thus, the CU-W1 XX family of airfoils was designed and numerically tested with the aid of XFOIL, resulting in larger aerodynamic efficiency compared with existing airfoils, such as the National Renewable Energy Laboratory’s (NREL) S8 series and Delft University of Technology’s ‘DU’ series. A single mention of airfoil noise is made related to the operating conditions but not as a design constraint.
Miller et al. presented a new family of flatback airfoils created by optimisation through a genetic algorithm in which the airfoil shapes were modified using curve parameterisation. The aerodynamic performance of the family of airfoils was evaluated with the aid of XFOIL code, and structural analysis was also considered in this case. Flatback airfoils may be found in the inner-to-mid region of the WT blades. They usually have a high resistance to flapwise bending and may attain high maximum lift coefficients. Thus, the CU-W1 XX family of airfoils was designed and numerically tested with the aid of XFOIL, resulting in larger aerodynamic efficiency compared with existing airfoils, such as the National Renewable Energy Laboratory’s (NREL) S8 series and Delft University of Technology’s ‘DU’ series. A single mention of airfoil noise is made related to the operating conditions but not as a design constraint.
Li et al. (2020)
Li et al. introduced a complex methodology for the design and optimisation of large HAWT airfoils for application in sites with prevalent low wind speed with high inflow turbulence. The parametric study considered aerodynamic and structural aspects of the problem and also included a noise emission assessment of the geometries by application of the NREL AirFoil Noise code (NAFNoise). Noteworthy are the special design considerations regarding sensitivity to inflow turbulence, with special attention to the small-scale turbulence, which has intensities recorded through anemometry in excess of 20%, although classic inflow turbulence intensity modelling in blade airfoil design is usually set to very low values. Having the NACA 63421 as the base airfoil, the authors conducted the optimisation process and obtained the CAS-W1-210 and CAS-Ti-210, which have presented better theoretical results for aerodynamic and aeroacoustic efficiency as evaluated by the XFOIL/RFOIL and NAFNoise analysis.
Li et al. introduced a complex methodology for the design and optimisation of large HAWT airfoils for application in sites with prevalent low wind speed with high inflow turbulence. The parametric study considered aerodynamic and structural aspects of the problem and also included a noise emission assessment of the geometries by application of the NREL AirFoil Noise code (NAFNoise). Noteworthy are the special design considerations regarding sensitivity to inflow turbulence, with special attention to the small-scale turbulence, which has intensities recorded through anemometry in excess of 20%, although classic inflow turbulence intensity modelling in blade airfoil design is usually set to very low values. Having the NACA 63421 as the base airfoil, the authors conducted the optimisation process and obtained the CAS-W1-210 and CAS-Ti-210, which have presented better theoretical results for aerodynamic and aeroacoustic efficiency as evaluated by the XFOIL/RFOIL and NAFNoise analysis.
Airfoil Families
Table 1 provides a summary of the main families of WT blade airfoils developed in the past two decades in a university environment, with their respective design and operational conditions.
Table 1 provides a summary of the main families of WT blade airfoils developed in the past two decades in a university environment, with their respective design and operational conditions.
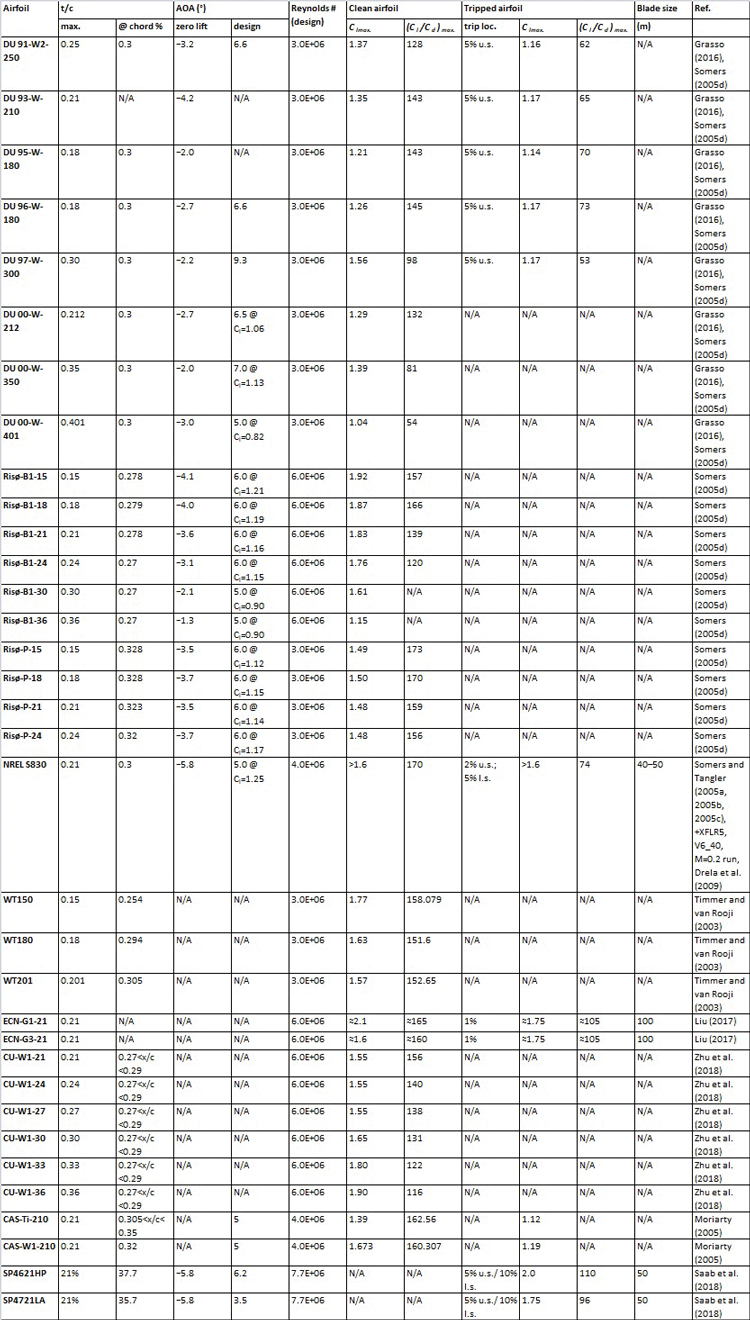
Table 1. Summary of major airfoils developed for large-size horizontal-axis wind turbines and their theoretical aerodynamic design characteristics
Notes:
1. While no coordinates of the airfoils described in Table are in the public domain, the coordinates of NREL’s ‘S’ family of airfoils are available for research purposes.
2. N/A = Not available.
3. t = airfoil thickness; c = airfoil chord; x = arbitrary position along the airfoil chord; AOA = angle of attack; Cl = airfoil lift coefficient; Cd = airfoil drag coefficient; u.s. = upper segment; l.s. = lower segment; M = Mach number.
1. While no coordinates of the airfoils described in Table are in the public domain, the coordinates of NREL’s ‘S’ family of airfoils are available for research purposes.
2. N/A = Not available.
3. t = airfoil thickness; c = airfoil chord; x = arbitrary position along the airfoil chord; AOA = angle of attack; Cl = airfoil lift coefficient; Cd = airfoil drag coefficient; u.s. = upper segment; l.s. = lower segment; M = Mach number.
References
- Guidati, G. and Wagner, S. 2000. Design of reduced noise airfoils for wind turbines. ECCOMAS 2000: European Congress on Computational Methods in Applied Sciences and Engineering, Barcelona, Spain.
- Fuglsang, P. and Bak, C. 2004. Development of the Risø wind turbine airfoils. Wind Energy 7(2), 145–162.
- Bertagnolio, F., Madsen, H. A. and Bak, C. 2009. Experimental validation of TNO trailing edge noise model and application to airfoil optimization. European Wind Energy Conference and Exhibition, Marseille, France.
- Cheng, J., Wang, Q., Zhang, S., Eecen, P. and Grasso, F. 2016. A new direct design method of wind turbine airfoils and wind tunnel experiment. Applied Mathematical Modelling 40(3), 2002–2014.
- Grasso, F. 2016. ECN-G1-21 Airfoil: design and wind-tunnel testing, Journal of Aircraft 53(5), 1478–1484.
- Saab Jr, J.Y., Pimenta, M.M., Faria A.M. and Rodriguez, S. 2018. Determination of local flow conditions and preliminary investigation on the validity of the PNoise code for large-size wind turbine. Journal of the Brazilian Society of Mechanical Sciences and Engineering 40(495), 493–504.
- Miller, M., Slew, K. and Matida, E. 2018. The development of a flatback wind turbine airfoil family. Wind Energy 21, 1372–1382.
- Li, X., Zhang, L., Song, J., Bian, F. and Yang, K. 2020. Airfoil design for large horizontal axis wind turbines in low wind speed regions. Renewable Energy 145, 2345–2357.
- D. M. Somers, ”The S825 and S826 Airfoils”, NREL/SR-500-36344, Golden, Co, 2005d.
- D. Somers and J. Tangler, ”The S822 and S823 Airfoils,” Golden, Co., 2005a.
- D. Somers and J. Tangler, ”The Airfoils S830, S831 and S832,” Golden, 2005b.
- D. Somers and J. Tangler, ”The Airfoils S833, S834 and S835,” Golden, Co., 2005c.
- M. Drela, H. Youngren and A. Deperrois, ”XFOIL/XFLR5”, Cambridge, Ma., 2009.
- W. Timmer and R. van Rooji, ”Summanry of the Delft University Wind Turbine Dedicated Airfoils”, Transactions of the ASME, Vol. 125, pp. 488-496, Nov. 2003. https://doi.org/10.1115/WIND2003-352.
- W. Zhu, W. Shen, E. Barlas, F. Bertagnolio and J. Sorensen, ”Wind turbine noise generation and propagation modeling at DTU Wind Energy: A review”, Renewable and Sustainable Energy Reviews, pp. 133-150, 2018. https://doi.org/10.1016/j.rser.2018.02.029.
- W. Liu, ”A review on wind turbine noise mechanism and de-noising techniques”, Renewable Energy, pp. 311-320, 2017. https://doi.org/10.1016/j.renene.2017.02.034.
- P. Moriarty, ”NAFNoise User’s Guide”, NREL, Golden, CO, 2005.
Biography of the Authors
Joseph Youssif Saab Jr is a full professor of energy and fluids and a senior member of the Poli-Wind research group.
Joseph Youssif Saab Jr is a full professor of energy and fluids and a senior member of the Poli-Wind research group.
Alexandre Martuscelli Faria is a mechanical engineer and a PhD candidate at Poli-USP. He currently works as a software developer and is a member of the Poli-Wind research group and co-developer of the PNoise module of the QBlade wind turbine blade design software.